GLYCOLYSIS
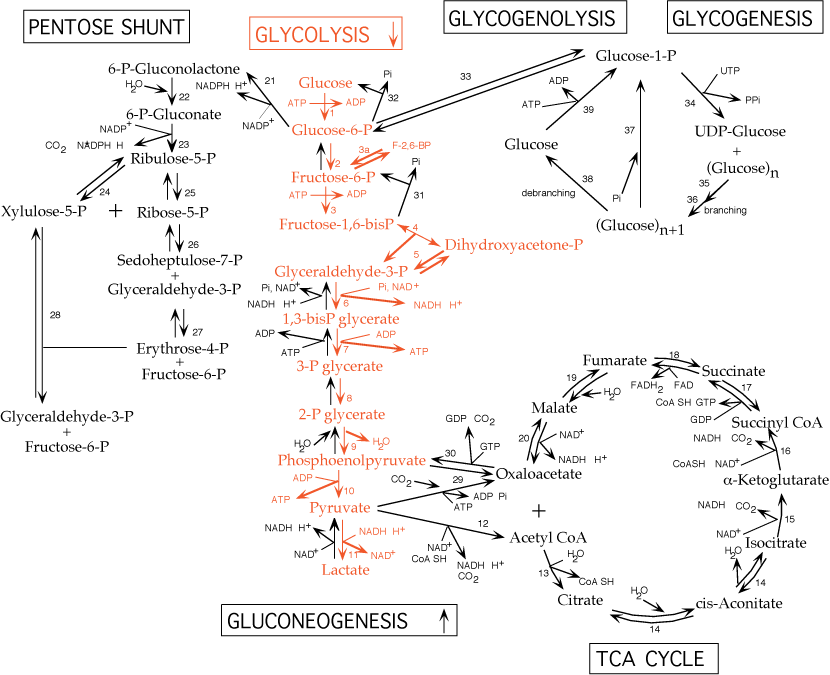
- Phosphorylation of glucose to form glucose-6-phosphate by hexokinase:
- irreversible, regulated step
- ATP is expended
- feedback inhibited by glucose-6-phosphate, the product of the reaction
- relatively low Km for glucose (about 0.1 mM)
- Glucose-6-phosphate, unlike glucose, is negatively charged, which prevents its exit from cells.
The liver and pancreatic β cells have a different hexokinase isoform called Glucokinase
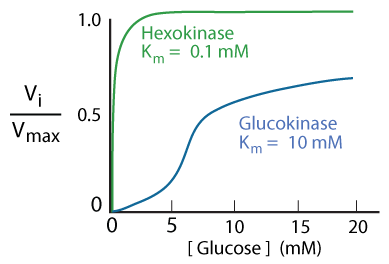
Glucokinase has a high Km (about 10 mM) and high Vmax for glucose, and is not product-inhibited. The liver does not require glucose as a fuel. Rather, it uses the carbons of glucose for the synthesis of triacylglycerols (fats), which are stored in adipose tissue, and it stores glucose as glycogen when blood glucose levels are high (e.g., after a meal), and recovers glucose from the stored glycogen for release into the blood when blood glucose is low (e.g., during fasting). The high Km , high Vmax , and inducibility of glucokinase allow the liver to capture glucose for storage as glycogen and to synthesize triacylglycerols when blood glucose levels are high, and to reduce the capture of glucose, thereby conserving it for other tissues that use it as a primary fuel (e.g., brain, heart, red blood cells, white muscle), when blood glucose levels are low. The non-product-inhibition of glucokinase allows the liver to capture glucose even when its intracellular levels of glucose-6-phosphate are high. Glucokinase gene transcription is repressed by glucagon, a hormone secreted by the pancreatic alpha cells in response to low blood glucose levels. When glucose levels are limited (low blood [glucose]) existing glucokinase is bound by the Glucokinase Regulatory Protein (GKRP), which inactivates and sequesters it in the cell nucleus, thereby preventing the glucose produced by gluconeogenesis and glycogenolysis from being rephosphorylated and trapped in the liver. Glucokinase gene transcription is stimulated by insulin, a hormone secreted by the pancreatic β cells in response to high blood glucose levels.
Glucokinase is a better sensor of glucose concentration than is Hexokinase because its sensitivity to glucose extends over a longer range of glucose concentration. Glucose sensing is important in pancreatic β cells in the release of insulin and in the liver, which switches from using glucose to producing glucose when blood glucose concentration is low. Several different mutations of the human glucokinase gene have been shown to be associated with Maturity-Onset Diabetes of the Young (MODY)
II. Glycolysis occurs in the cytosol, yielding 2 ATP, 2 pyruvate and 2 (NADH + H+) from each glucose molecule.The glycolytic pathway is present in all cells and has a central role in generating ATP with and without oxygen. One mole of glucose is converted to two moles of pyruvate and two moles of NADH. The pathway uses energy from the oxidation of glucose to generate ATP by substrate-level phosphorylation (transfer of phosphate from a high-energy intermediate to ADP).
Click The Image
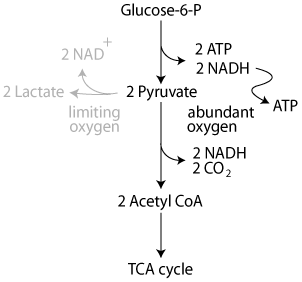
In the presence of oxygen (aerobic glycolysis) NADH electrons are passed to oxygen in the electron transport chain, thereby allowing the reoxidization of NADH to NAD+, and ATP production. Pyruvate is oxidized completely to CO2 and water.
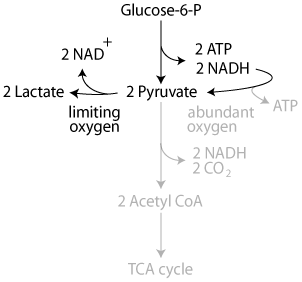
When oxygen is limiting (anaerobic glycolysis) NADH electrons are passed to pyruvate, reducing it to lactate and regenerating NAD+. ATP is not produced in the electron transport chain.
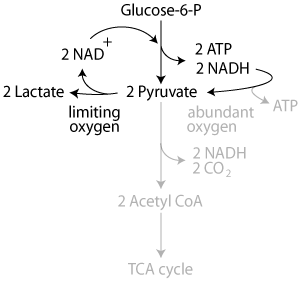
The regenerated NAD+ allows glycolysis, which requires NAD+, to proceed even though oxygen, which, during aerobic glycolysis would accept the NADH electrons, is absent.
In the first phase of glycolysis glucose-6-phosphate is converted to a form that can be readily cleaved into two phosphorylated three-carbon units. In the second phase, high-energy phosphate (as ATP) is generated from these two three-carbon units.
- Isomerization of glucose-6-phosphate (aldose) to fructose-6-phosphate (ketose) by phosphoglucose isomerase:
- freely interconverted
- Phosphorylation of fructose 6 phosphate to form fructose-1,6-bisPhosphate by phosphofructokinase 1 (PFK1):
THIS IS THE RATE-LIMITING STEP OF GLYCOLYSIS AND THE MOST IMPORTANT SITE OF REGULATION.
- irreversible, regulated step
- ATP is expended
- responds to cell energy charge ([ATP]/[AMP] ratio), allosteric inhibition by ATP is antagonized by AMP
- ATP lowers the affinity (increases the Km) of the enzyme for its substrate, fructose-6-phosphate, thereby slowing glycolysis at high cellular [ATP]/[AMP]
- Citrate, an early TCA cycle intermediate, also inhibits PFK1, by increasing its sensitivity to ATP. A high level of citrate indicates that alternate fuels such as fatty acids and ketone bodies (discussed in later lectures) are available
- inhibited by low pH, thereby guarding against excessive lactate formation from glycolysis, which would cause a further drop in blood pH (lactic acidosis)
- activated allosterically by fructose 2,6-bisPhosphate which increases the affinity for fructose 6-phosphate (lowers the Km) and decreases the sensitivity to ATP inhibition
- Phosphorylation of Fructose 6 phosphate to form Fructose 2,6-bisPhosphate by Phosphofructoskinase 2 (PFK2), a remarkable protein with TWO, distinct active sites (bifunctional enzyme). One site has phosphofructose kinase activity (Phosphofructokinase 2 (PFK2)) and the other site has fructose bisPhosphatase activity (Fructose 2,6-bisPhosphatase (FBPase)).
- Fructose 2,6-bisPhosphate is the most important regulator of glycolysis in the liver. Its abundance is a reflection of whether blood glucose levels are high or low (see below).
- "Feed-forward" allosteric activation by Fructose 6-phosphate
- The kinase reaction is favored by the dephosphorylated form of the bifunctional enzyme.
- The phosphatase reaction is favored by the phosphorylated form of the bifunctional enzyme. This is NOT a reversal of the kinase reaction (don't re-create ATP).
- The liver bifunctional enzyme is phosphorylated by protein kinase A (cAMP-dependent protein kinase).
- cAMP levels rise in response to signaling by hormones such as glucagon, epinephrine and norepinephrine, or in response to events that inhibit cAMP phosphodiesterase (e.g., caffeine intake). Inactive protein kinase A consists of two catalytic subunits and a cAMP-binding regulatory subunit. The catalytic subunits are inactive when bound to the regulatory subunit, which dissociates from the catalytic subunits when it binds cAMP (see “Hormone Regulation” in the top menu).
- Glucagon, secreted by pancreatic alpha cells in response to low blood [glucose], results in an increase of liver cAMP, which causes the release of the catalytic subunits from the repressive regulatory subunit; the active protein kinase A phosphorylates the liver bifunctional enzyme, resulting in a decrease of Phosphofructokinase 2 activity and an increase of Fructose 2,6-bisPhosphatase activity, thereby preventing build-up of Fructose 2,6-bisPhosphate and, thus, slowing liver glycolysis and conserving blood glucose at step #3 (Phosphofructokinase 1).
- Conversely, when blood [glucose] is high, glucagon levels are low, insulin levels are high, the liver bifunctional enzyme is dephosphorylated, synthesis of Fructose 2,6-bisPhosphate is favored, and liver glycolysis is increased at enzymatic step #3 (Phosphofructokinase 1).
Click The Image
ATP, citrate, and low pH inhibit PFK I. AMP and Fructose 2,6-bisPhosphate stimulate PFK I. Fructose 2,6-bisPhosphate is the most important regulator in the liver; it is an intracellular reporter of systemic [glucose]. ATP and AMP are the most important regulators of skeletal muscle glycolysis.
Relatively small amounts of Fructose 6-Phosphate are converted to Fructose 2,6-bisPhosphate by phosphofructokinase II (PFK II). PFKII activity is activated in response to increased glucose and insulin signaling. Consequently, glycolysis in the liver increases.
Decreased [glucose], which results in decreased insulin and increased glucagon, causes the deactivation of PFK II and the activation of Fructose 2,6-bisPhosphatase, which converts Fructose 2,6-bisPhosphate to Fructose 6-Phosphate, thereby slowing glycolysis in the liver and conserving glucose when [glucose] is low. Fructose 6-Phosphate stimulates PFK II and inhibits Fructose 2,6-bisPhosphatase.
Fructose 2,6-bisPhosphate lowers the Km of Phosphofructokinase 1. The concentration of F-2,6-bisPhosphate depends on the relative activity of Phosphofructokinase II and Fructose 2,6-bisPhosphatase.
3a.
In summary, the Phosphofructokinase kinase 2 activity predominates in the "fed" state, Fructose 2,6-bisPhosphatase activity predominates in the "fasted/starved" state.
- Muscle does not have glucagon receptors.
- The muscle isoforms of the PFK2/FBPase bifunctional enzyme are not phosphorylated (not regulated) in response to the fasted/starved state.
- Skeletal and cardiac muscle do have β2 adrenergic (epinephrine) receptors.
- The skeletal muscle bifunctional enzyme isoform has no phosphorylation site - it is produced by differential splicing and does NOT have the phosphorylation site that is phosphorylated by protein kinase A in the liver. Epinephrine signaling causes skeletal muscle cell glycogen breakdown to yield increased glucose, which the skeletal muscle uses for glycolysis in situations of acute stress. Because the muscle isoform of the bifunctional enzyme is not phosphorylated, muscle glycolysis is not down regulated in response to epinephrine signaling; skeletal muscle, thus, gets a boost of glucose from the degradation of its glycogen for the production of energy via glycolysis and the subsequent complete oxidation of glucose carbon in the TCA cycle with the generation of electrons that are passed to the electron transport chain for the production of ATP to provide energy for skeletal muscle to take action in response to an existential acute stress (Epinephrine/adrenalin is known as the “fight or flight” hormone; it signals the body to prepare for action needed to respond to an acute stress).
- The skeletal muscle bifunctional enzyme isoform is regulated mainly by Fructose 6 phosphate, which activates the Phosphofructokinase 2 activity and inhibits the Fructose 2,6-bisPhosphatase activity. As Glucose 6-phosphate is converted to Fructose 6 phosphate by the reversible enzyme Phosphoglucose Isomerase (reaction #2), when the level of Glucose 6 phosphate is increased, the level of Fructose 6 phosphate is also increased.
- In the liver, as in skeletal muscle, glycogen is degraded in response to signaling by epinephrine through the β2 adrenergic receptors. However, in contrast to the skeletal muscle bifunctional enzyme, the liver bifunctional enzyme is phosphorylated by activated protein kinase A in response to epinephrine signaling, thereby causing a decrease in the bifunctional enzyme Phosphofructokinase 2 activity and an increase in the Fructose 2,6-bisPhosphatase activity, resulting in a decrease of liver glycolysis due to the decreased level of Fructose 2,6-bisPhosphate. The glucose produced from the breakdown of liver glycogen is, therefore, not used for glycolysis by the liver, but is rather exported to the blood to supply extra-hepatic tissue with an extra source of energy to respond to an existential acute stress.
- The cardiac muscle isoform of the bifunctional enzyme is produced from a different gene than that in liver and skeletal muscle. It has a phosphorylation site located near its carboxy terminus, which is different from the phosphorylation site located at the N-terminus in the liver bifunctional enzyme isoform. Under normal conditions, cardiac muscle predominantly uses fatty acids and lactate as its major energy sources. Phosphorylation of the cardiac muscle bifunctional enzyme isoform in response to epinephrine/adrenalin signaling acting through cardiac β 2 adrenergic receptors and protein kinase A causes a decrease of the cardiac bifunctional enzyme Fructose 2,6-bisPhosphatase activity and an increase in the Phosphofructokinase 2 activity, which raises the concentration of Fructose 2,6-bisPhosphate, causing an increase in the activity of phosphofructokinase 1, thereby increasing cardiac glycolysis to supply increased energy for increased heart contractions during an existential acute stress signaled by epinephrine/adrenalin The increased heart contractions cause an increase in blood flow throughout the body to carry extra nutrients and oxygen to tissues that respond to an existential acute stress.
The respective skeletal muscle and cardiac muscle bifunctional enzymes are regulated differently than the liver bifunctional enzyme.
Click The Image
Regulation Of The Liver Bifunctional Enzyme
Abundant Glucose - Phosphofructokinase 2 Predominates
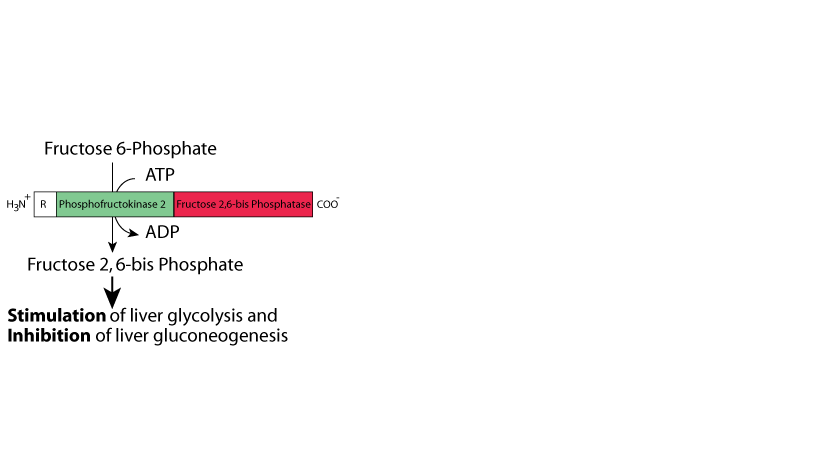
When glucose is abundant the liver bifunctional enzyme is not phosphorylated and the Phosphofructokinase 2 activity predominates. Fructose 2,6-bisPhosphate is synthesized and it stimulates glycolysis (and inhibits gluconeogenesis - see Gluconeogenesis).
Regulation Of The Liver Bifunctional Enzyme
Decreased Glucose - Fructose 2,6-bisPhosphatase Predominates
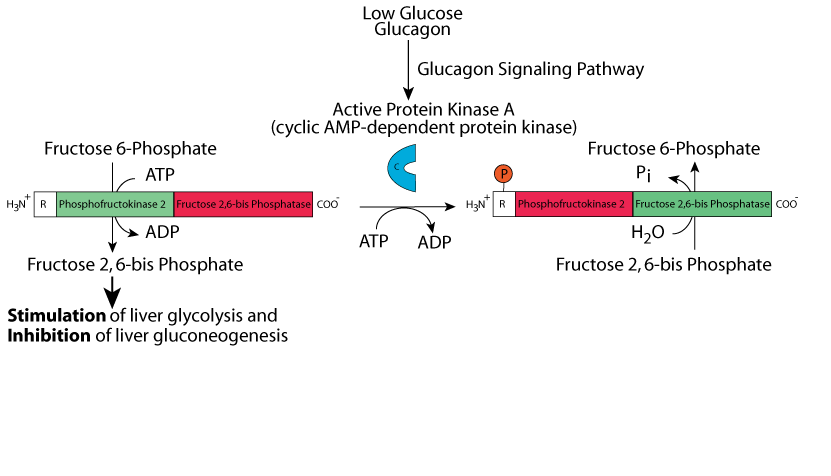
Phosphorylation of the liver bifunctional enzyme by protein kinase A (cyclic AMP-dependend protein kinase) in response to glucagon signaling when [glucose] is low causes the activity of Phosphofructokinase 2 to decrease and the activity of Fructose 2,6-bisPhosphatase to increase. Fructose 2,6-bisPhosphate is degraded and liver glycolysis decreases.
Regulation Of The Liver Bifunctional Enzyme
Increased Glucose - Phosphofructokinase 2 Predominates
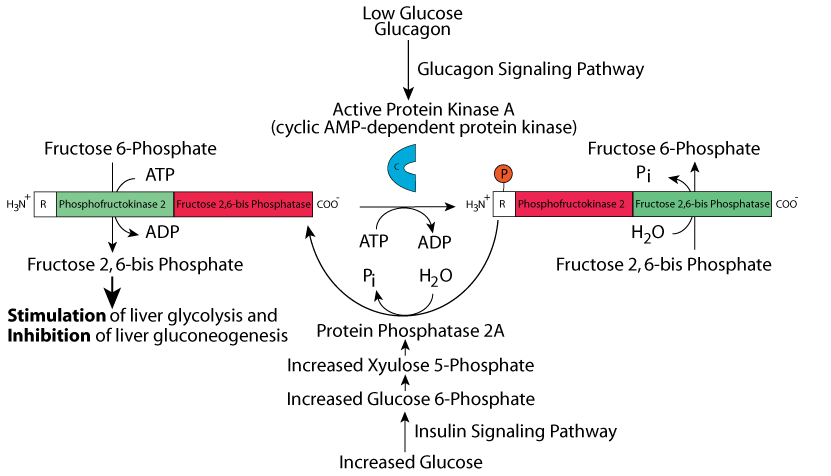
When [glucose] increases, some glucose is converted to Xyulose 5-Phosphate (see the non-oxidative phase of the Pentose Phosphate Pathway) whose concentration increases. Xyulose 5-Phosphate activates Protein Phosphatase 2A, which removes the phosphate group from the bifunctional enzyme, lowering the activity of Fructose 2,6-bisPhosphatase and increasing the activity of Phosphofructokinase 2. Fructose 2,6-bisPhosphate is synthesized and it stimulates liver glycolysis by increasing the activity of Phosphofructokinase 1.
Regulation Of The Liver Bifunctional Enzyme
Epinephrine Signaling - Fructose 2,6-bisPhosphatase Predominates
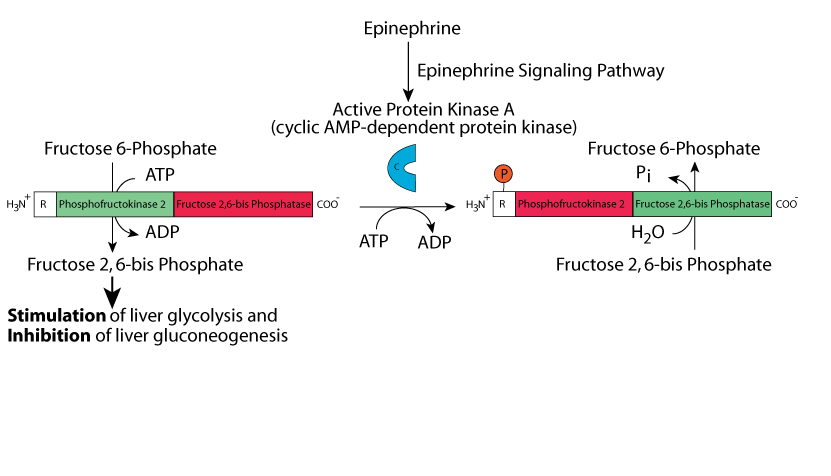
Epinephrine/adrenaline stimulates liver cell β2 adrenergic receptors, causing an increase in cyclic AMP, which activates Protein kinase A. Protein kinase A phosphorylates the liver bifunctional enzyme, causing a reduction in Phosphofructokinase 2 activity and an increase in Fructose 2,6, bisPhosphatase activity, therby reducing [Fructose 2,6-bisPhosphate], decreasing the activity of Phosphofructokinase 1, and reducing liver glycolysis.
Regulation Of The Skeletal Muscle Bifunctional Enzyme
Normal [Fructose 6 Phosphate] - Phosphofructokinase 2 Predominates
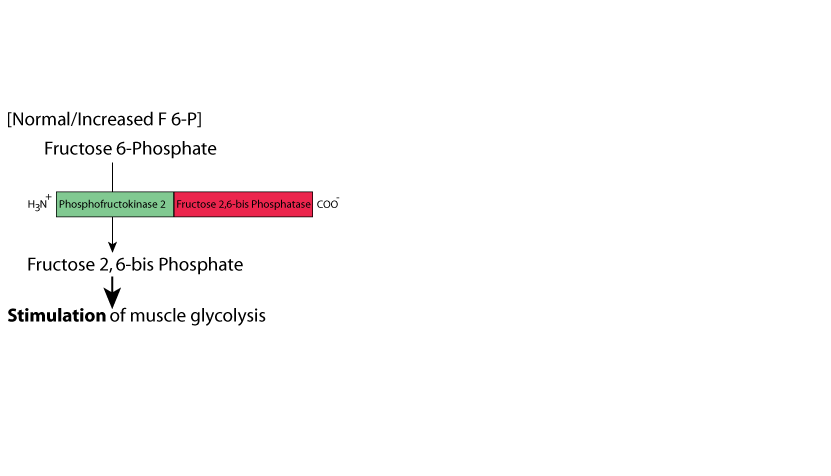
The skeletal muscle bifunction enzyme isoform is mainly regulated according to the level of Fructose 6-Phosphate, which is in equilibrium with Glucose 6-Phosphate by a reversible reaction catalyzed by Phosphoglucose Isomerase (reaction #2). Normal [Fructose 6-Phosphate] stimulates the activity of the Phosphofructokinase 2 activity, and inhibits the Fructose 2,6-bisPhosphatase activity, thereby stimulating glycolysis at Phosphofructokinase 1. Skeletal muscle does not have glucagon receptors. Skeletal muscle does have β2 adrenergic receptors which signal in response to epinephrine/adrenaline signaling to activate protein kinase A. However, the skeletal muscle bifunctional enzyme isoform is a splice variant of the liver bifunctional enzyme that DOES NOT HAVE A PHOSPHORYLATION SITE. Therefore, skeletal muscle protein kinase A does not phosphorylate the muscle bifunctional enzyme in response to epinephrine/adrenaline signaling. Epinephrine/adrenaline signaling through the β2 adrenergic receptors on skeletal muscle cells results in the degradation of skeletal muscle glycogen to generate glucose phosphate to provide incerased energy that skeletal muscle cell uses to respond (i.e., “Fight or Flight”) to an existential acute stress.
Regulation Of The Skeletal Muscle Bifunctional Enzyme
Reduced [Fructose 6 Phosphate] - Fructose 2,6-bisPhosphatase Predominates
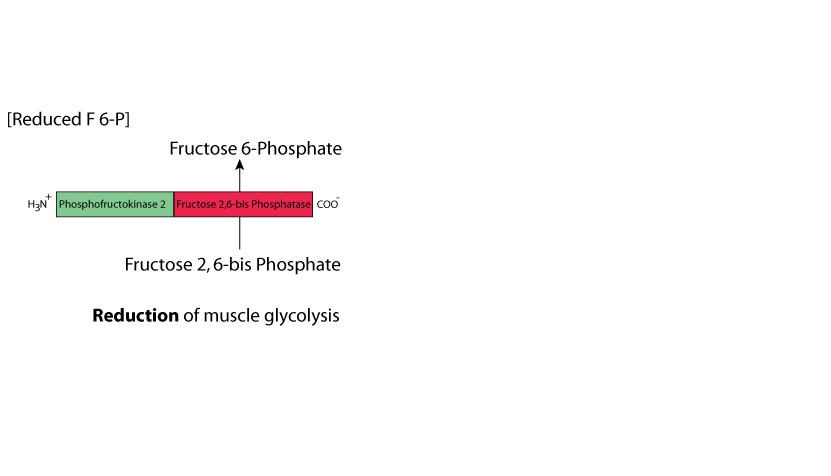
When [Fructose 6-Phosphate] falls below the normal value (for example, when blood [glucose] is lower than normal), the skeletal muscle bifunctional enzyme Phosphofructokinase 2 activity decreases and Fructose 2,6-bisPhosphatase activity increases, thereby reducing [Fructose 6-Phosphate] and skeletal muscle glycolysis decreases. When blood [glucose] is lower than normal, alternative fuels (fatty acids, ketone bodies) become available that skeletal muscle can use for the generation of ATP. Skeletal muscle suppresses the use of alternative fuels when sufficient glucose is available.
Regulation Of The Cardiac Muscle Bifunctional Enzyme
Normal Function - Fructose 2,6-bisPhosphatase Predominates
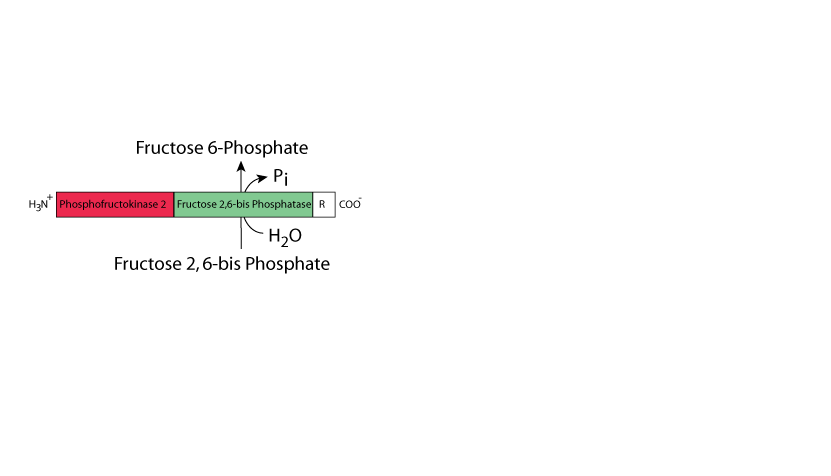
The cardiac muscle isoform of the bifunctional enzyme is produced from a different gene than that in liver and skeletal muscle. Under normal conditions, cardiac muscle predominantly uses fatty acids and lactate as its major energy sources. The cardiac muscle bifunctional enzyme Fructose 2,6-bisPhosphatase predominates.
Regulation Of The Cardiac Muscle Bifunctional Enzyme
Epinephrine/Adrenaline Signaling - Phosphofructokinase 2 Predominates
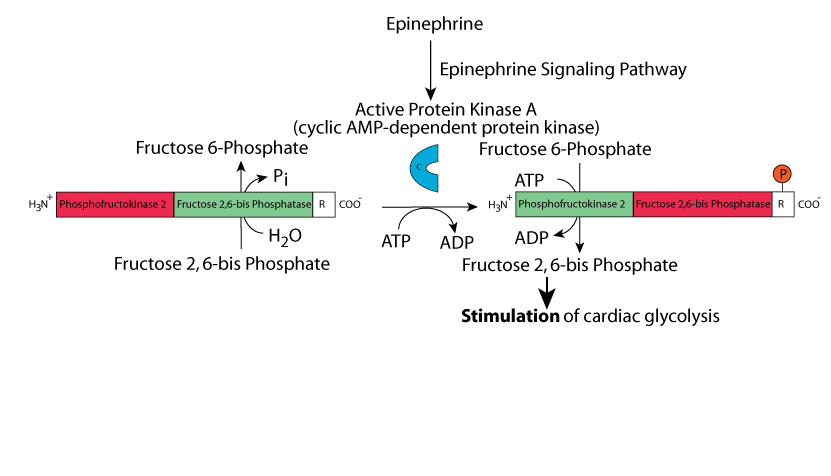
Signaling by epinephrine/adrenaline through cardiac muscle β2 adrenergic receptors results in phosphorylation of the cardiac muscle bifunctional enzyme isoform at a site different from that which is phosphorylated in the liver bifunctional enzyme isoform. In contrast to phosphorylation of the liver bifunctional enzyme isoform in which Fructose 2,6-bisPhosphatase activity predominates, Phosphofructokinase 2 activity predominates in the phosphorylated cardiac bifunctional enzyme isoform. The resulting increased [Fructose 2,6-bisPhosphate] causes an increase in cardiac muscle glycolysis by stimulating the activity of cardiac Phosphfructokinase 1 to provide increased energy to power the increase contraction rate of cardiac muscle in response to an existential acute stress signaled by epinephrine/adrenaline.
- Cleavage of fructose-1,6-bisPhosphate to form dihydroxyacetone phosphate and glyceraldehyde 3 phosphate [G3P] by aldolase:
Synthesis of glycerol 3-phosphate in adipose tissues:- reversible
- G3P is on the direct pathway of glycolysis
- several isozymes of aldolase (aldolases A, B, C, and fetal aldolase) are active against fructose 1,6-bisPhosphate
- dihydroxyacetone phosphate is the substrate for the synthesis of the glycerol 3-phosphate required by adipose tissue for the esterification of fatty acids in the production of stored triacylglycerols (fat); because adipose tissues do not have glycerol kinase, they rely on glycolysis to supply dihydroxyacetone phosphate , which they reduce to glycerol 3-phosphate
- insulin signaling results in GLUT 4 mobilization from adipose cytosolic membranes to the adipose plasma membrane for the capture of the glucose to be used in the glycolytic pathway for the production of dihydroxyacetone phosphate
- Interconversion of dihydroxyacetone phosphate and glyceraldehyde 3 phosphate by triose phosphate isomerase:
- reversible
- Oxidation of glyceraldehyde 3-phosphate to form 1,3-bisPhosphoglycerate
(1,3-BPG), a high-potential phosphorylated compound, by glyceraldehyde 3-phosphate dehydrogenase:
Red blood cells use 2,3-bisPhosphoglycerate Mutase to convert 1,3-bisPhosphoglucerate to 2,3 bisPhosphoglycerate (2,3-BPG). 2,3-BPG binds to the central cavity of hemoglobin, lowering the affinity of hemoglobin for oxygen. Oxygen is more readily released when hemoglobin contains 2,3-BPG. Fetal hemoglobin does not bind 2,3-BPG, allowing transfer of oxygen from adult hemoglobin to fetal hemoglobin.
- reversible
- ATP NOT expended — part of the energy obtained in the oxidation of G3P is harvested as an electron pair donated to NAD+ — under anaerobic conditions the resulting NADH is recycled back to NAD+ by donating its electrons to pyruvate at reaction #11, under aerobic conditions it will donate its electrons to oxygen in the production of ATP by oxidative phosphorylation, becoming reoxidized back to NAD+
- Carbon 1 of 1,3 BPG is at the oxidation level of a carboxylic acid. The phosphoryl group of this mixed anhydride has high transfer potential.
- 1,3-bisPhosphoglycerate is converted to form large amounts of
2,3-bisPhosphoglycerate in red blood cells by 2,3-bisPhosphoglycerate mutase. 2,3-bisPhosphoglycerate regulates the hemoglobin/O2 dissociation curve − deficiency of glycolytic enzymes can cause hemolytic anemia.
- Transfer of the high-energy phosphate bond from
1,3-bisPhosphoglycerate to form ATP and 3 phosphoglycerate by phosphoglycerate kinase: - reversible
- substrate-level phosphorylation; ATP is produced – this and the preceding reaction (#6) allow for a net gain of ATP from glycolysis — particularly important in tissues with no (red blood cells) or few (e.g., renal medulla) mitochondria or low oxygen
- Interconversion of 3 phosphoglycerate and 2 phosphoglycerate by phosphoglycerate mutase:
- reversible
- Dehydration of 2 phosphoglycerate to form phosphoenolpyruvate [PEP] by enolase:
- reversible
- the dehydration elevates the transfer potential of the phosphoryl group
- Transfer of high-energy phosphate bond from phosphoenolpyruvate to form ATP and pyruvate by pyruvate kinase:
- essentially irreversible, highly regulated step
- substrate-level phosphorylation; ATP is produced
- "feed forward" activation by fructose 1,6-bisPhosphate
- liver isozyme shows cooperative phosphoenolpyruvate binding, allosteric inhibition by ATP and alanine (an amino acid synthesized from pyruvate in one metabolic step)
- inhibition by glucagon-triggered phosphorylation − this slows glycolysis in the liver when blood [glucose] is low, thereby conserving glucose for use by tissues that use it as a primary fuel. Recall that PFK2 (step #3) is also phosphorylated, and its kinase activity is reduced, by the same mechanism, in response to low blood glucose levels.
- muscle isozyme is NOT regulated by phosphorylation
- A rare, hyperactive mutant pyruvate kinase is harmful to red blood cells because it depletes the precursor pool, diverting substrate from the synthesis of 2,3-bisPhosphoglycerate, thereby impairing hemoglobin function
- Regeneration of NAD+ through reduction of pyruvate (the electron acceptor) to form lactate by lactate dehydrogenase (fermentation — the generation of ATP by a process in which both the electron donor and the electron acceptor are organic molecules):
- reversible
- Major option used by cells under anaerobic conditions to regenerate cytosolic NAD+. Without O2, mitochondria can't resupply NAD+ — this step allows glycolysis to continue anaerobically by regenerating the NAD+ required in step #6. Anaerobic glycolysis is obviously necessary in red blood cells, which lack mitochondria. It is also important when the oxygen supply is limiting, e.g., in exercising muscle, or anoxia due to impaired blood supply, as in stroke.
- abdominal (central) obesity,
- elevated blood pressure,
- elevated fasting plasma glucose,
- high serum triglycerides, and
- low high-density cholesterol (HDL) levels.
Click The Image
Cori Cycle
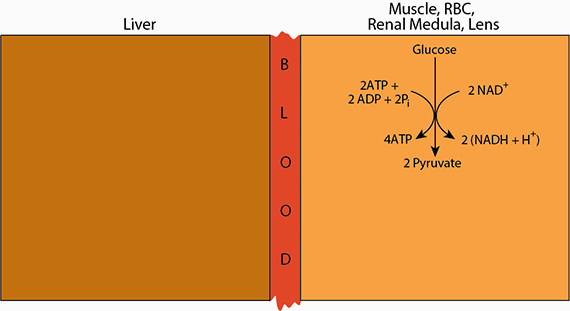
Pyruvate (ionized pyruvic acid) is the oxidized end product of glycose produced by glycolysis. Under areobic conditions, the electrons collected as NADH are transferred from the NADH to electron carriers, which transfer them to the mitochondrial electron transport chain. The pyruvate is, likewise, transferred to the mitochondria for complete oxidation to CO2.
Cori Cycle
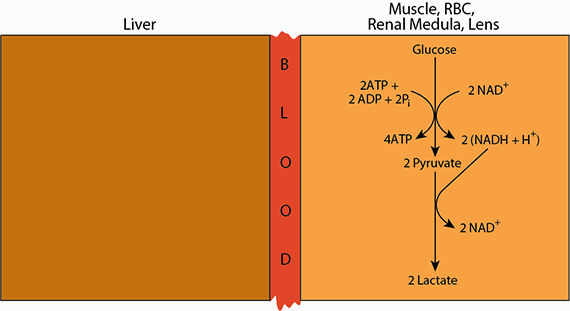
When oxygen is limiting (anaerobic conditions), electron carriers and intermediates of the electron transport chain remain reduced, as electrons are unable to be offloaded to oxygen, the ultimate electron acceptor. As a result, glycolysis slows or comes to a halt because the cytosolic NAD+ is reduced to NADH and cannot accept more electrons in the oxidative reaction of glycolsis (reaction 6, glyceraldehyde 3-phosphate dehydrogenase). Under these anaerobic conditions cytosolic NADH is used to reduce pyruvate to lactate, thereby regenerating NAD+and allowing glycolysis to continue.
Cori Cycle
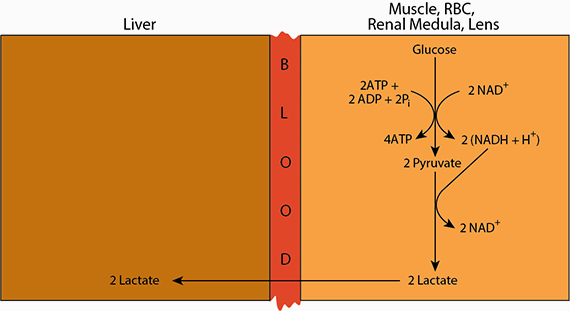
Lactate is transferred via the blood from peripheral tissues to the liver.
Cori Cycle
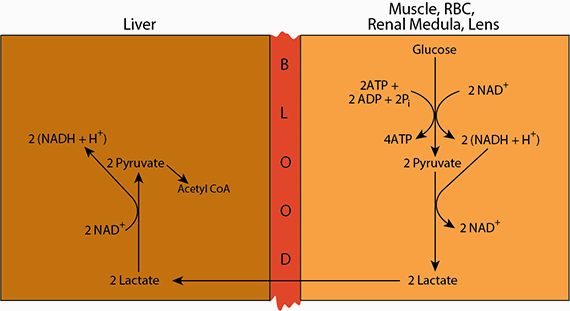
In the liver, the lactate is re-oxidized to pyruvate, which may enter the mitochondria and become oxidized in the TCA cycle to yield energy, or ...
Cori Cycle
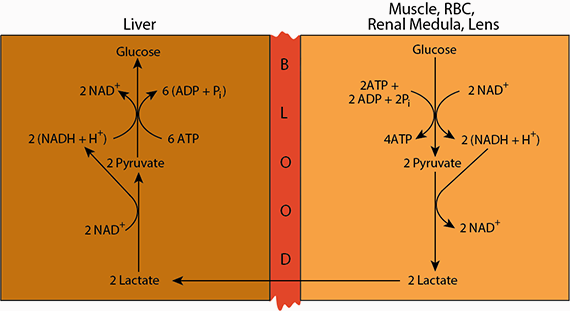
if the blood glucose level is low, the pyruvate enters the gluconeogenic pathway in which it is coverted to glucose.
Cori Cycle
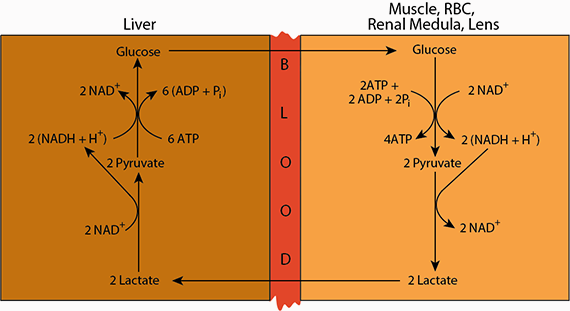
Finally, the glucose resulting from gluconeogenesis is released from the liver into the blood to supply tissues that rely on glucose as an energy soure.
The Fate of Lactate (ionized lactic acid)
Lactic acid released from cells undergoing anaerobic glycolysis is taken up by other tissues (primarily liver, heart and skeletal muscle) and oxidized back to pyruvate (ionized pyruvic acid), which is then oxidized to CO2 in the TCA cycle. In the liver, the lactate is likewise oxidized back to pyruvate, which can either be oxidized to CO2 in the TCA cycle, or, when blood [glucose] is low, used to synthesize glucose (glyconeogenesis), which is returned to the blood. The cycling of lactate and glycose between peripheral tissues and the liver is called the “Cori Cycle” after its discoverers, Carl Ferdinand Cori and Gerty Cori, who, in 1947, were awarded the Nobel Prize in Medicine or Physiology for their work on carbohydrate metabolism, including the elucidation of the Cori Cycle (also known as the "Lactate Cycle"). Summary of aerobic glycolysis (including hexo-/glucokinase):
Summary of anaerobic glycolysis (including hexo-/glucokinase):
Phosphofructokinase 1 is inhibited by low pH. The lactate (ionized lactic acid; lactate + H+ at physiological pH; pKa of lactic acid = 3.85, physiological pH = 7.4) that is formed under anaerobic conditions contributes to lower pH. Therefore, slowing glycolysis at the committed step slows lactate production and helps prevent acidosis (lactic acidosis) due to excess lactate (lactic acid).
Metabolism of Galactose
Galactose occurs in many foods, especially in dairy products in which lactose is its main dietary source. It also occurs in certain pectins, gums and mucilages. Other sources include sugar beets, a large number of fruits, including apples, bananas, cherries, and grapes, and in vegetables, including broccoli, carrots, cabbage, cauliflower, tomatoes, peas, pumpkins and spinach.
The enzymes for the conversion of galactose to glucose 1-phosphate are present in many tissues, including the adult erythrocyte, fibroblasts and fetal tissues. The liver has a high activity of these enzymes and can convert dietary galactose to blood glucose and glycogen. The metabolism of galactose is higher in infants than in adults. Newborns ingest up to one gram of galactose (as lactose) per kilogram during feeding, but the rate of galactose metabolism is so high that the concentration in the blood remains low.
Click The Image
Galactose metabolism
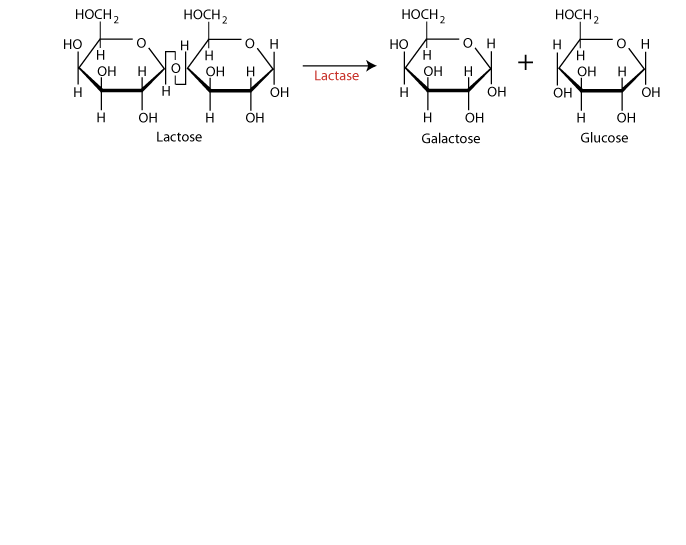
Lactose, a disaccharide of galactose and glucose, is a major source of galactose. Lactase, produced by the cells that line the small intestine, converts lactose to its two monosaccharide components.
Galactose metabolism
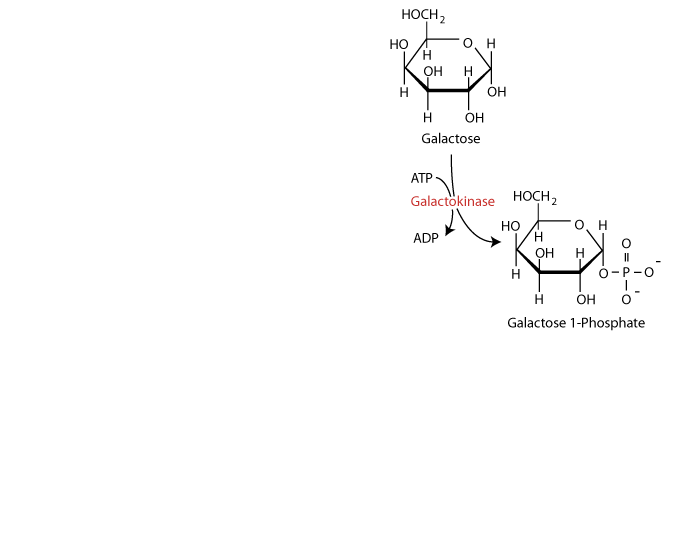
Dietary galactose is metabolized principally by conversion to glucose 1-phosphate. As for glucose, galactose requires phosphorylation for entry into cellular metabolism. However, unlike glucose, which is phosphorylated at carbon 6, galactose is phosphorylated at carbon 1 by the enzyme galactokinase to yield galactose 1-phosphate.
Galactose metabolism
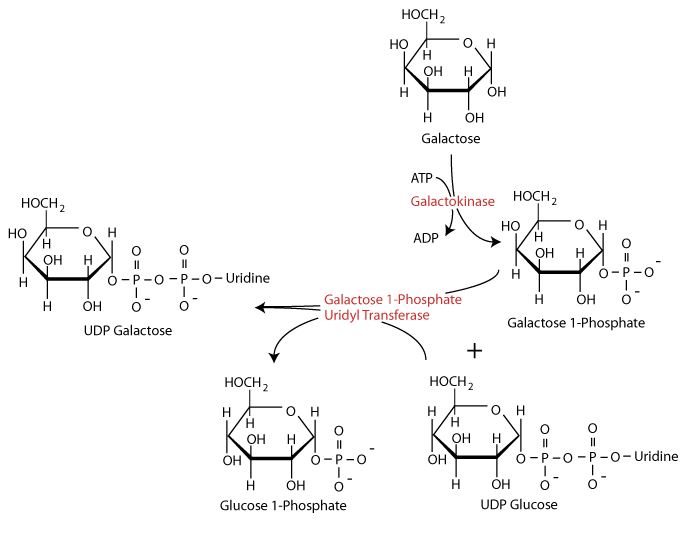
Galactose 1-phosphate then receives a uridine monophosphate group from uridine-diphosphate glucose (UDP-glucose) with the release of glucose 1-phosphate in a reaction catalyzed by the enzyme galactose 1-phosphate uridyl transferase. Genetic deficiency of the uridyl transferase (primary galactosemia) results in inappropriate metabolism of galactose to toxic forms such as galactitol. If untreated, galactosemia may be fatal.
Galactose metabolism
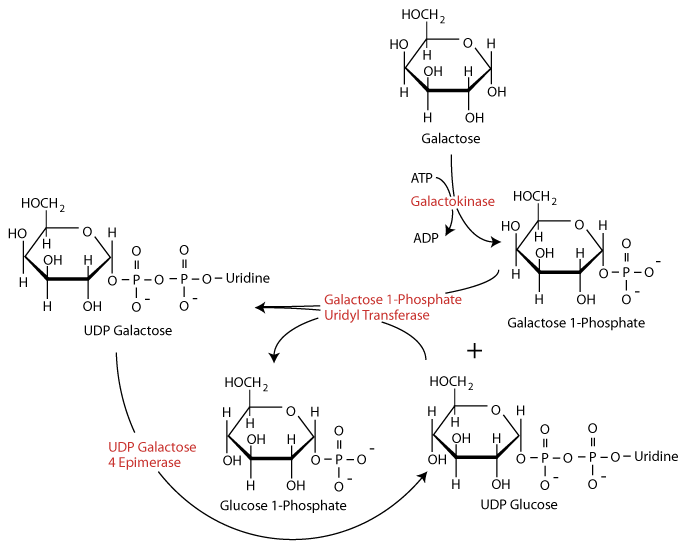
The enzyme UDP Galactose 4-epimerase acts to regenerate UDP-glucose.
Galactose metabolism
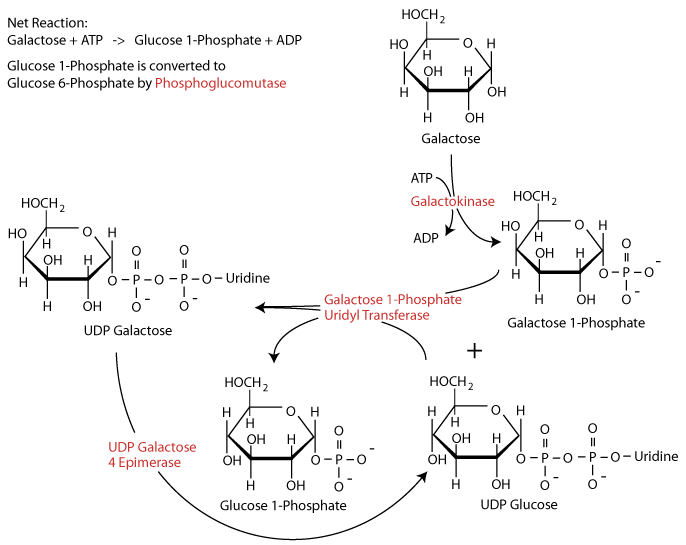
Glucose 1-phosphate is converted to glucose 6-phosphate by the enzyme phosphoglucomutase.
Metabolism of Fructose

Click The Image
Fructose metabolism
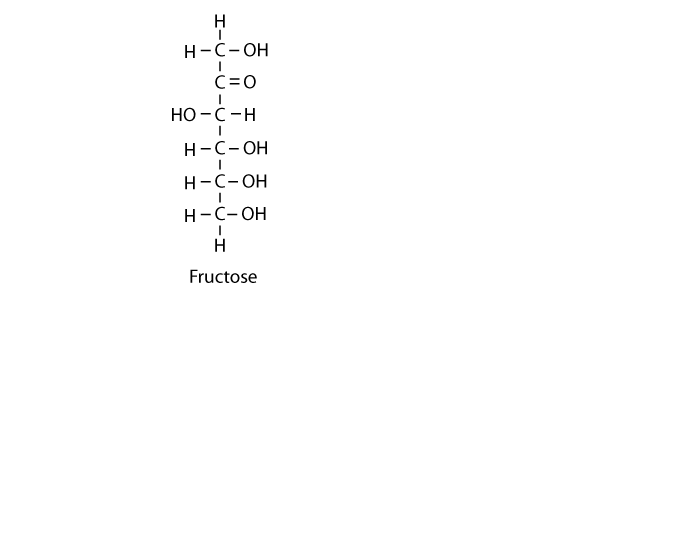
The principal dietary sources of fructose are sucrose — a disaccharide of glucose and fructose — and monosaccharide fructose added to a multitude of processed foods, including high fructose corn syrup, a significant additive to soft drinks. In addition, the polyol pathway converts glucose to fructose.
Fructose metabolism
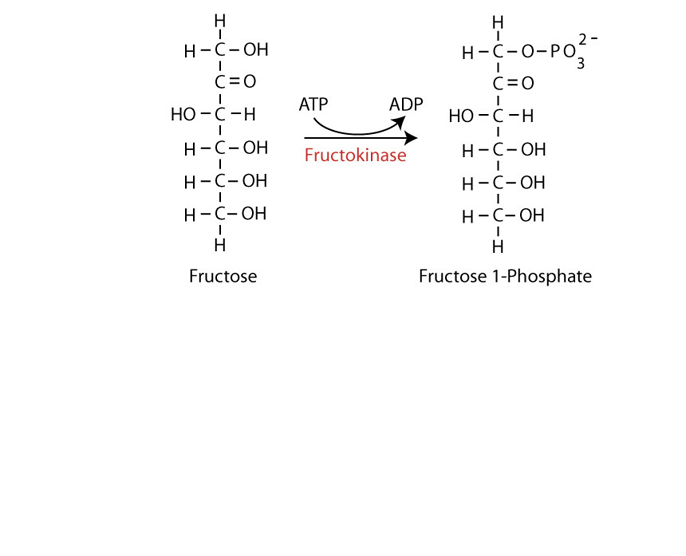
As for both glucose and galactose, fructose must be phosphorylated to enter cellular metabolism Phosphorylation at carbon 1 is catalyzed by the enzyme fructokinase (ketohexokinase)) to yield fructose 1-phosphate, The liver gets a large proportion of the dietary fructose. Liver fructokinase C isozyme (ketohexokinase C) metabolizes fructose to fructose 1-phosphate so rapidly that it can transiently deplete intracellular phosphate and ATP.
Fructose metabolism
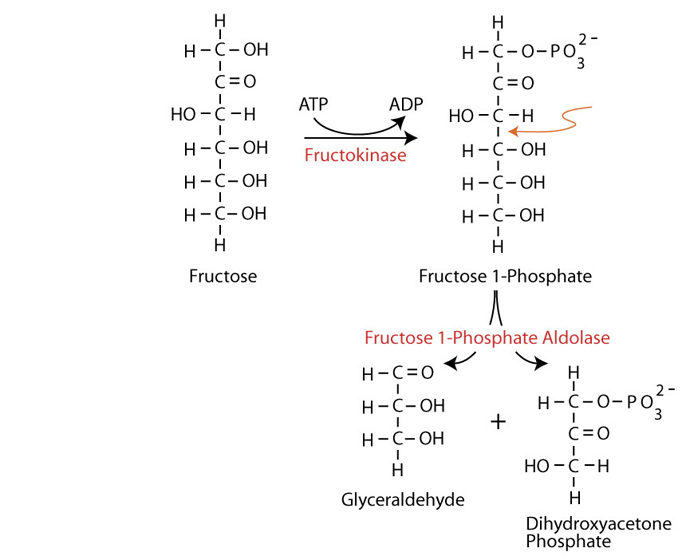
Fructose 1-phosphate is cleaved by the enzyme fructose 1-phosphate aldolase (aldolase B) – the only one of four aldolase isozymes able to cleave fructose 1-phosphate) to dihydroxyacetone phosphate (an intermediate of glycolysis) and glyceraldehyde.
Fructose metabolism
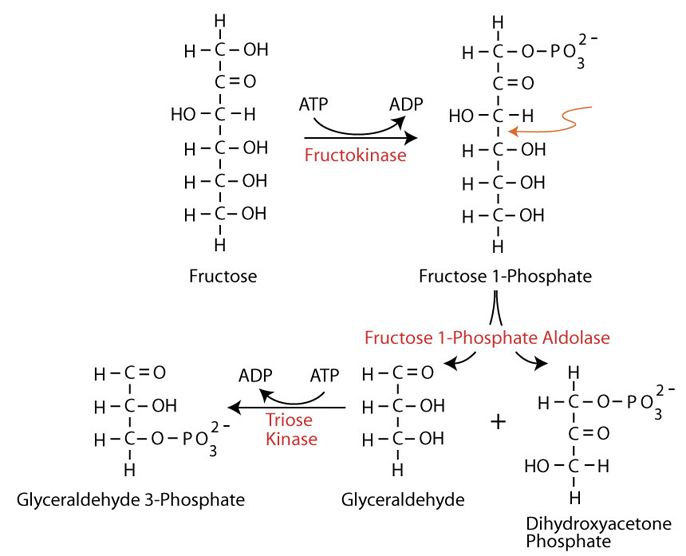
The glyceraldehyde is phosphorylated by the enzyme triose kinase to yielding glyceraldehyde 3-phosphate (an intermediate of glycolysis). Dihydroxyacetone phosphate is converted to glyceraldehyde 3-phosphate by Triose Phosphate Isomerase (#5).
Fructose-1-phosphate aldolase (aldolase B) cleavage of fructose-1-phosphate is the rate-limiting step in fructose metabolism. The enzyme has a low affinity for fructose 1-phosphate and is very slow at physiological fructose 1-phosphate concentrations. Consequently, after ingestion of a high dose of fructose, normal individuals accumulate fructose 1-phosphate in the liver. Individuals with hereditary fructose intolerance (HFI), due primarily to a deficiency of fructose 1-phosphate aldolase, accumulate much higher amounts than normal of fructose 1-phosphate in their livers. Prolonged fructose ingestion in these infants leads ultimately to ultrastructural changes in the liver and kidney, resulting in hepatic and/or renal failure and, potentially, death.
HFI is usually a disease of infancy because adults with fructose intolerance who have survived avoid eating fruits, table sugar and other sweets. Symptoms of HFI include vomiting, convulsions, irritability, poor feeding as a baby, hypoglycemia, jaundice, hemorrhage, hepatomegaly, hyperuricemia and potentially kidney failure. While HFI is not clinically a devastating condition, there are reported deaths in infants and children as a result of the metabolic consequences of HFI. Death in HFI is always associated with problems in diagnosis.
Sugar and Fatty Liver Disease
Fructose has been associated with fatty liver since Pliny the Elder noted that the famous Roman chef, Marcus Apiciu, would make fatty liver (foie gras) by feeding geese dates (a rich source of fructose). Subsequently, Justus von Liebig the German chemist, made the observation that simple carbohydrates stimulated fat accumulation in the liver. By the 1960s numerous scientists reported that fructose was distinct from glucose in its unique ability to increase both plasma triglycerides and liver fat.
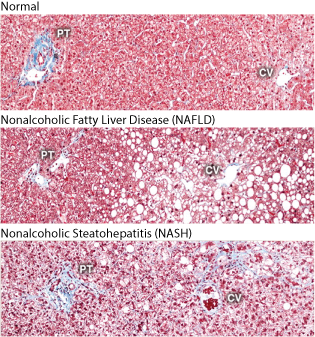
Fructose intake from added sugars (mainly sucrose and/or high fructose corn syrup (HFCS)) correlates with the epidemic rise in obesity, metabolic syndrome, nonalcoholic fatty liver disease (NAFLD), and nonalcoholic steatohepatitis (NASH); NASH is NAFLD that progresses to become associated with inflammation, and diabetes.
Dietary fructose, sucrose, or HFCS have been shown to have a special tendency to induce fatty liver, as well as inflammation, in experimental animals. It usually requires at least 8–24 weeks on a high fructose diet to develop a fatty liver, with more progressive disease the longer the exposure. Often the administration of fructose also induces other features of metabolic syndrome, including elevated blood pressure, elevated serum triglycerides, and insulin resistance. In part, the fatty liver may be due to increased energy intake. However, if diet is controlled so that the control group animals ingest the same amount of total energy, fructose-fed rats still develop features of metabolic syndrome, although weight gain is not different between fructose-fed and control animals. It is even possible to induce fatty liver with a calorically restricted diet if the diet is high in sugar, and it has also been reported that a high fructose diet can induce fatty liver in the absence of weight gain.
Question:
Why is fructose, as compared with glucose, particularly conducive to the production of fat?See Answer
For a recent New York Times article on the increasing U.S. incidence of NASH click the link below. Note that a mutation in the gene that encodes adiponutrin (PNPLA3) increases the risk of developing NASH.
Increasing Nonalcoholic Steatohepatitis
Glucose In The Clinic
Click The Image
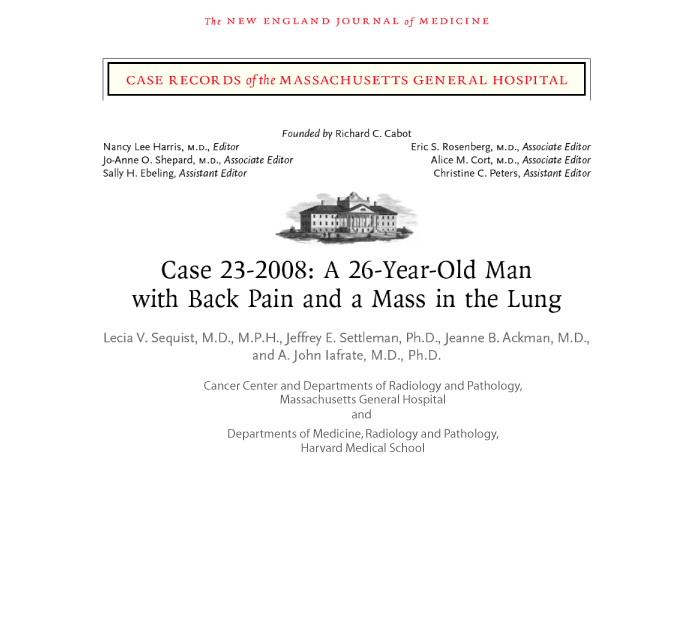
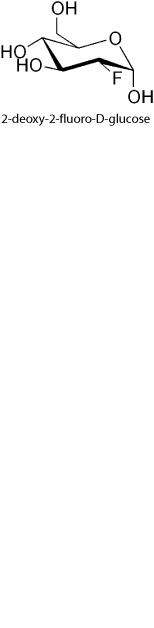
18Fluorine is a fluorine radioisotope that is an important source of positrons. It has a mass of 18.000938 and its half-life is 109.77 min. It decays by positron emission 97% of the time and electron capture 3% of the time. Both modes of decay yield stable 18oxygen. Because 18fluorine is easily introduced to compounds with hydroxyl groups and its half-life is relatively long, 18fluorine is often incorporated in diagnostic drugs for use in PET scans.
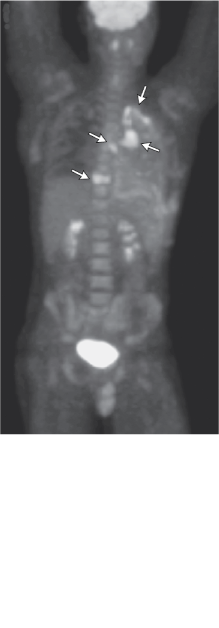
The increased use of glucose by some solid tumors, even when they are well oxygenated (i.e., vascularized) and performing aerobic glycolysis, the "Warberg Effect", allows them to be imaged by PET scan.
An 18fluorodeoxyglucose positron-emission tomographic study on hospital day 4 shows markedly increased tracer uptake in the left suprahilar mass, left apical pleural thickening, subcranial lymphadenopathy, and the T9 vertebral body (arrows).
The kidneys and the bladder are also imaged, as the 18fluorodeoxyglucose is excreted in the urine because, although it is taken up by cells, it is not metabolized due to the substitution of a fluorine for a hydroxyl at carbon 2.
The brain is also prominently imaged, not because of tumorigenicity, but because the brain is a heavy user of glucose as its source of energy - the brain uses approximately 3/4 of the human daily glucose requirement.
Genes encoding glycolytic enzymes are ubiquitously overexpressed in 24 cancer classes.
Cancer cells upregulate glycolysis to maintain energy production in the absence of oxygen (Pasteur Effect) early in tumor development, when the tumor is not vascularized.
Eventually glycolysis becomes the preferred energy production pathway, even during reoxygenation (Warberg Effect) as the tumor induces the growth of new blood vessels.
The Warburg effect is the enhanced conversion of glucose to lactate (ionized lactic acid) observed in tumor cells, even in the presence of normal levels of oxygen.
Otto Heinrich Warburg demonstrated in 1924 that cancer cells show an increased dependence on glycolysis to meet their energy needs, regardless of whether they were well-oxygenated or not. Converting glucose to lactate, rather than metabolizing it through oxidative phosphorylation in the mitochondria, is far less efficient, as less ATP is generated per unit of glucose metabolized. Therefore, a high rate of glucose uptake is required to meet increased energy needs to support rapid tumor progression.
Increasing amounts of H+ due to lactic acid production and pumped into the extracellular space to maintain normal intracellular pH create an acidic microenvironment, which is known to select for cells with enhanced metastatic potential as well as provide resistance to chemotherapeutic agents.
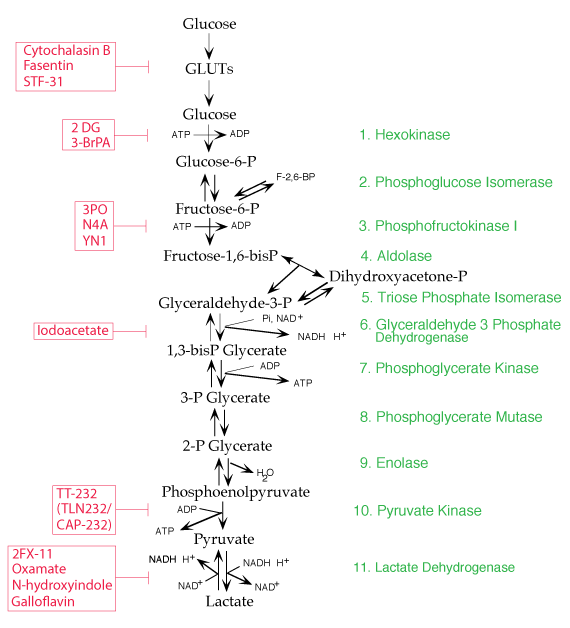
Several drug agents are currently being tested for use in combination with approved anticancer drugs in an attempt to slow glycolysis in tumor cells in order to limit the production of lactate (lactic acid) and the acidic tumor microenvironment it creates. The purpose is to prevent the development of the acidic microenvironment that reduces the efficacy of anticancer drugs and promotes tumor metastasis.